By William P. Owen1, William M. Dalrymple2, and John G. Diehl3
1California Department of Transportation, Sacramento, California, [email protected]
2California Department of Transportation, Sacramento, California, [email protected]
3GEOVision Geophysical Services Inc., Corona, California, [email protected]
ABSTRACT
In February 2017, failure of the Oroville Dam Main Spillway, and near failure of the Emergency Spillway, resulted in the precautionary emergency evacuation of nearly 200,000 people downstream of the facility. Response and recovery efforts for the disaster were far-ranging and geophysical investigations were included as part of those efforts. Both state government and private consultant resources were deployed for those tasks. Ground penetrating radar, refraction tomography and borehole geophysical logging were used to assist with determining condition of the remaining Main Spillway, the variability of the bedrock weathering profile, and rock velocity at critical locations across the facility. The data assisted the facility engineering team regarding operational decisions immediately post-failure, and longer term, with design of the replacement structures.
INTRODUCTION
Lake Oroville is the second largest artificial reservoir in California (Figure 1). Completed in 1967, the dam at Oroville is the tallest in the United States, impounding up to 3.5 million acre-feet of water. After several years of drought, 2017 produced the second wettest winter on record in California at that time. On the morning of February 7, 2017, a significant portion of the service spillway failed during routine discharge of the reservoir. The resulting crisis forced the dam operators to quickly make critical and difficult decisions, where neither the risk trade-offs nor the results of those decisions could be predicted with complete confidence. The chain of events that ultimately occurred resulted in the overtopping of the Emergency Spillway for the first time in its history. This caused extensive erosion, with head-cutting migrating upstream to the base of the Emergency Spillway, jeopardizing its integrity. The risk of failure was sufficient to warrant emergency evacuation of nearly 200,000 people downstream until the crisis could be contained.
The emergency response to the failure was far-ranging, and although geophysical surveys were only a small part of the total effort, the information from those surveys was important to key decisions in the repairs for the damaged structures. Both state and contract resources were employed to acquire condition data on the surviving portion of the Main Spillway, and contract services were deployed to acquire seismic tomography, borehole televiewer and downhole velocity data in support of the foundation repair for the Emergency Spillway. Time-critical deadlines for information required rapid turnaround of the geophysical data as it was collected. Many users of that data were not regular consumers of geophysics. That, combined with the need for rapid dissemination, focused data presentation on graphic and visual representations that could quickly convey the most important pieces of information needed to assist the engineers and geologists responsible for making time-critical decisions.
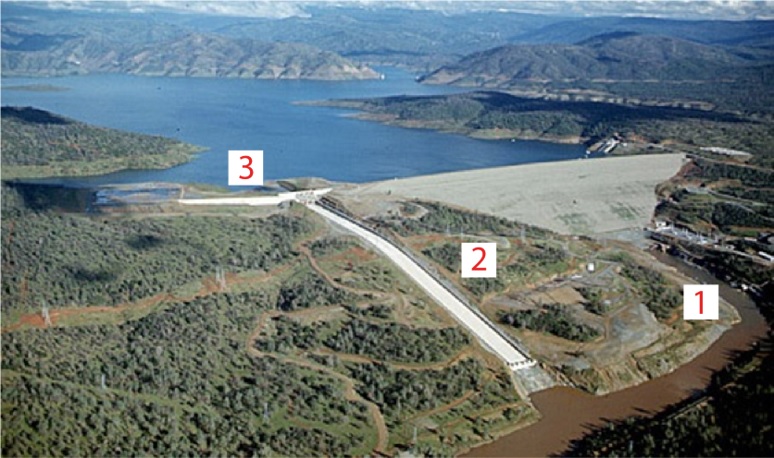
Figure 1. Lake Oroville Dam and Reservoir, circa 2015 (before spillway failure). Outflows from the reservoir are controlled from three locations: the power plant (1), the Main Spillway (2), and the Emergency Spillway (3).
PROLOGUE
2017 was the second wettest winter on record in California. Heavy January rains in the Oroville drainage basin were causing the reservoir to quickly rise. Under normal operations, this was a manageable situation, and spillway releases at that time were well within design limits. That changed on the morning of February 7, when shortly after 10:00 AM, employees reported a substantial disturbance in water flow at the lower half of the spillway (Figure 2). The trunnion gates were closed by 12:25 pm, and inspection revealed that a significant portion of the spillway
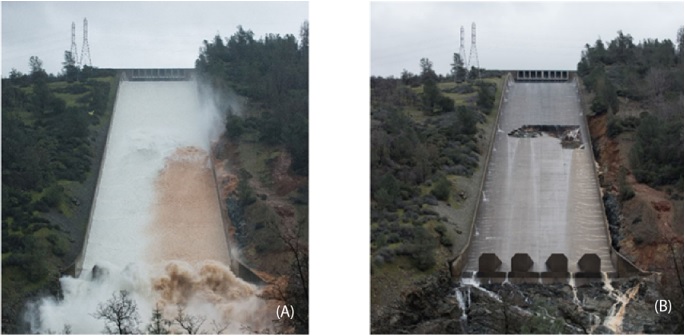
Figure 2. The Main Spillway (A) at time of first damage, February 7, and (B) shortly after shutdown showing initial damage.
slab had washed away, forming a large erosion hole where the slab sections were now missing. As inspections continued, lake waters continued to rise. The damaged Main Spillway had to be reopened, but outflows were reduced to minimize further damage. Operators knew further damage was certain, but the extent could not be predicted, and further erosion of the foundation rock and loss of additional slab sections occurred during this period. By that time, the majority of discharge flow was no longer confined to the spillway and was now channelized to the southeast, causing rapid and severe erosion of soil and rock south of the spillway (Figure 3).

Figure 3. Views of the Main Spillway (A) during post-failure managed emergency discharge in February 2017, and (B) shortly after shutdown for emergency repairs in March 2017. Note the extreme erosional damage from flow channelization at the right (southeast) side of the image.
By February 10, reservoir inflows exceeded the established combined discharge capacities of the power plant and the crippled Main Spillway. On February 11, the Emergency Spillway at Oroville was activated for the first time in its history. Although erosion was anticipated downstream of the Emergency Spillway, the extent could not be fully predicted. The uncontrolled discharge caused erosion and channelization as it flowed down the unprotected natural terrain, with head-cutting regressing upstream rapidly toward the base of the spillway (Figure 4). This threatened the stability of the Emergency Spillway structure itself, so at 3:44 pm on February 12, an evacuation order was issued for about 188,000 downstream residents. Although reservoir drawdown ultimately succeeded and the evacuation order was lifted on February 14, the area remained under an evacuation warning for an additional five weeks. On February 27, the spillway gates were closed, and on-site investigations and recovery actions commenced. During that time, investigations and remedial actions were interrupted occasionally for service spillway releases to manage the reservoir.
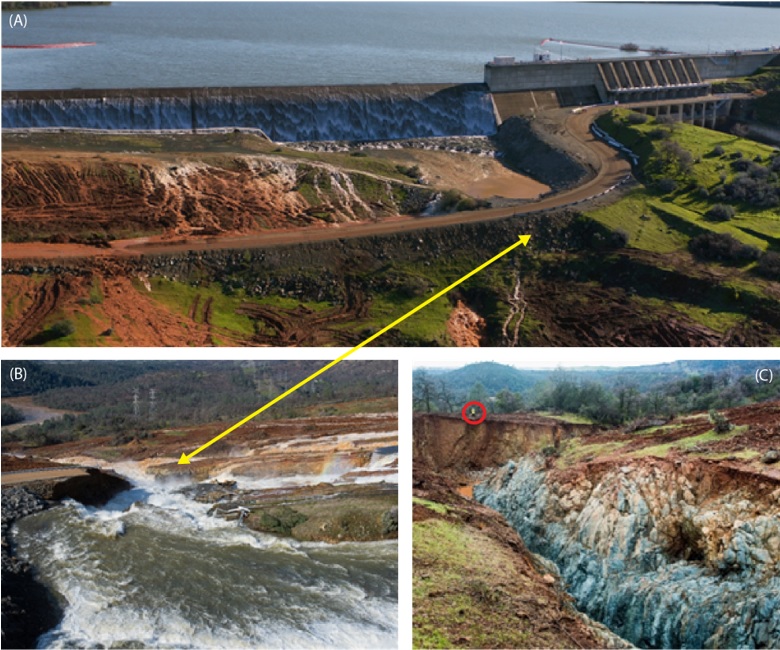
Figure 4. Views at the Emergency Spillway (A) during overtopping on February 11, (B) downstream view from the crest during peak flows, showing washout of the access road (arrow indicates relative location), (C) post-flow, near the former access road, showing extent of downstream erosion and channelization (Photo by author; note the human inspector in the background [circled] for scale).
GEOPHYSICS EFFORTS
State government geophysicists and private consultants were used to acquire geophysical data during the recovery efforts. State resources were limited to the Main Spillway, consultant efforts were broader in scope and included the Main and Emergency spillways and adjacent areas. We’ll limit our discussion to the state efforts at the Main Spillway, and the consultant work on the Emergency Spillway.
Main Spillway
Under Mutual Aid agreements established and administered by the Governor’s Office and the California Office of Emergency Services, all agencies of the state must provide emergency management personnel and technical specialists to support disaster operations. On March 2, 2017, the Office of Emergency Services requested assistance from the Geophysics and Geology Branch at the California Department of Transportation (Caltrans). A Caltrans crew arrived on site the same day to perform ground penetrating radar (GPR) investigations on the remaining portion of the undamaged Main Spillway. Over the course of eight days, Caltrans personnel acquired a linear equivalent of 27.2 miles of GPR data in support of the repair efforts.
Investigation goals for the Caltrans GPR work required us to: 1) evaluate the location and distribution of the drainage gallery beneath the undamaged portion of the spillway, 2) provide estimates of concrete thickness within the undamaged spillway, 3) identify any potential voids at the base of concrete within the undamaged spillway, and if possible, 4) identify the distribution of any rock discontinuities beneath the undamaged spillway
Our work was complicated by the nature of the emergency response. Due to the extremely short response time frame, the investigation plan had to be developed literally overnight. We didn’t know how much time we had to complete work. Reservoir levels were not yet stable and emergency evacuation of the spillway could occur at a moment’s notice. If that happened, all equipment would be abandoned. We also had to share space with other crews who were frantically racing to make repairs ahead of planned spillway reactivation, which required work staging to accommodate changing priorities.
Initial information available for investigation planning were:
- Minimum design concrete thickness was 15 inches,
- Concrete reinforcement was a single layer of #5 rebar on a 12”x12” grid,
- The spillway slabs were secured by rock anchors embedded five feet into rock and spaced at roughly ten-foot intervals (Figure 5),
- 4’x4’ reinforcing mesh was embedded within the concrete, about 4 inches above rock surface and placed at the base of each rock anchor,
- At the base of concrete, a sub-drain gallery of 6-inch vitreous clay pipe (VCP; the same material used in clay pots) with gravel filter was arranged in a herringbone pattern spaced at about 25-foot intervals (Figure 5). Drainage relied on passive gravity flow, so pipe elevation decreased from the center of the spillway toward collector pipes outside the Main Spillway.
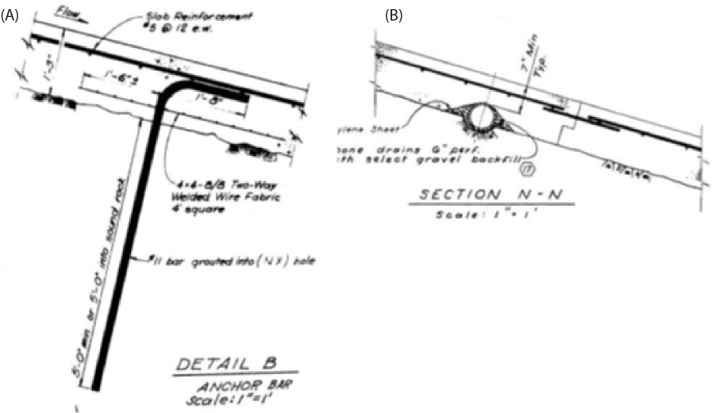
Figure 5. Construction details for the concrete slab, anchors and subdrains for the Main Spillway. (A) Anchor details showing welded wire mesh fabric above top of rock. (B) Cross-section showing vitreous clay pipe (VCP) drain with gravel filter pack at the base of concrete. From DWR (1965).
The spillway foundation is located within the Smartville Ophiolite Complex (Cole and McJunkin, 1978; Saucedo and Wagner, 1992). Rock here consists of steeply-dipping, foliated, metamorphosed volcaniclastics, pillow basalts, breccia, and diabase dikes and sills. Rock is blue-grey on fresh surfaces, grading to dark brown where weathered. It is very hard where fresh, but is strongly foliated and exhibits steeply dipping tight to open joints. The thickness of the weathering profile is quite variable, on the order of 10-100 feet. The dielectric properties of the Oroville bedrock proved to be very advantageous for GPR acquisition. With a 500 MHz transducer, depth of investigation was at least 6 feet, with reflections from 8 feet or greater observed at many locations. We observed reflections from joints or fractures in the underlying rock. Many of those reflections were good enough to measure joint attitudes.
We generated multiple maps with different data views of the entire undamaged spillway section. We used Google Earth 2016 aerial imagery for the base map. Those images were pre-failure and were the best available at that time. Using Google Earth imagery for base maps provided a common file format with easily understood visual references. “Rubber-sheeting” of the imagery was accurate to about six inches, more than sufficient for data presentation. Image resolution was good enough to recover project stationing from visible concrete joints and known landmarks.
The subdrains were conspicuous on the GPR profiles (Figure 6), and the herringbone pattern of the drains and gravel filter were readily visible in plan view (Figure 7A). These features are artificially induced voids, so they gave us some idea of how a void beyond the drains should appear. We also observed reflections from the lower reinforcing mesh (Figure 7A), and ringing off the vertical rebar of the rock anchors was seen at most locations (Figure 6).
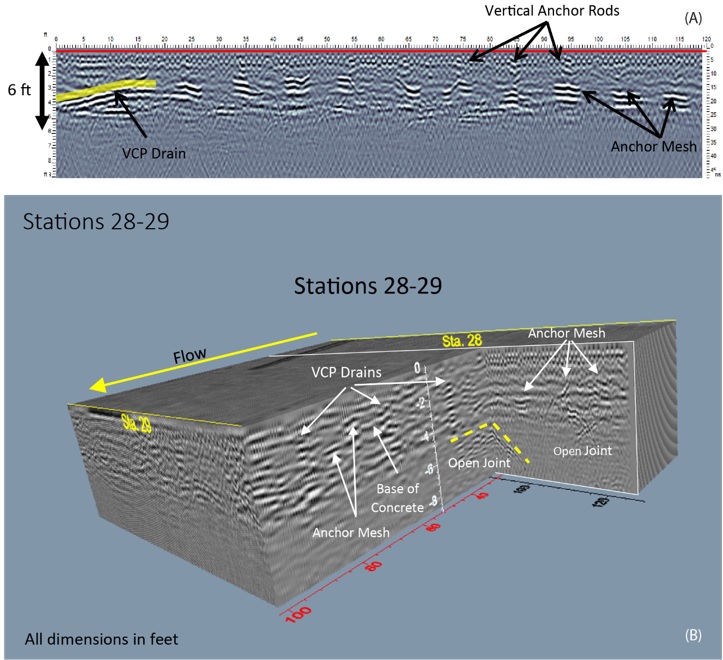
Figure 6. GPR data views in the vicinity of Station 28-29. (A) Profile view. (B) 3D cutaway view (composited from successive 2D profiles). Examples of interpreted features labeled as shown. Note lack of consistent reflection from base of concrete. VCP drains and anchor mesh served as proxies for estimating concrete thickness. From Owen and Mallah (2017).
Conspicuously absent in the data was any consistent reflection from the base of the concrete. We simply didn’t find any strong evidence of voids or delamination between the base of concrete and the top of rock along the remaining spillway, with the exception of an area in the vicinity of Station 26 (Figure 7D). That observation was consistent with an area of “significant clay fines” at the top of the rock surface noted during construction, and confirmed during demolition of the spillway during replacement the following year (IFT, 2018). Figure 7D includes the extent of clay fines observed during spillway demolition overlaid on the GPR interpretations. Figure 8B includes a photo taken of that exposed rock surface during demolition.
The ability to observe reflections several feet into rock allowed us to collect some information on the rock discontinuities immediately beneath the spillway. It was even possible to get attitudes from many of the rock features observed on the profiles. Although the rock discontinuities were a low-priority target for our investigation, we could produce a rapid, qualitative assessment of the distribution of observed discontinuities by plotting high-contrast images of the rock reflections, creating a composite image of reflections from the rock joints and fractures beneath
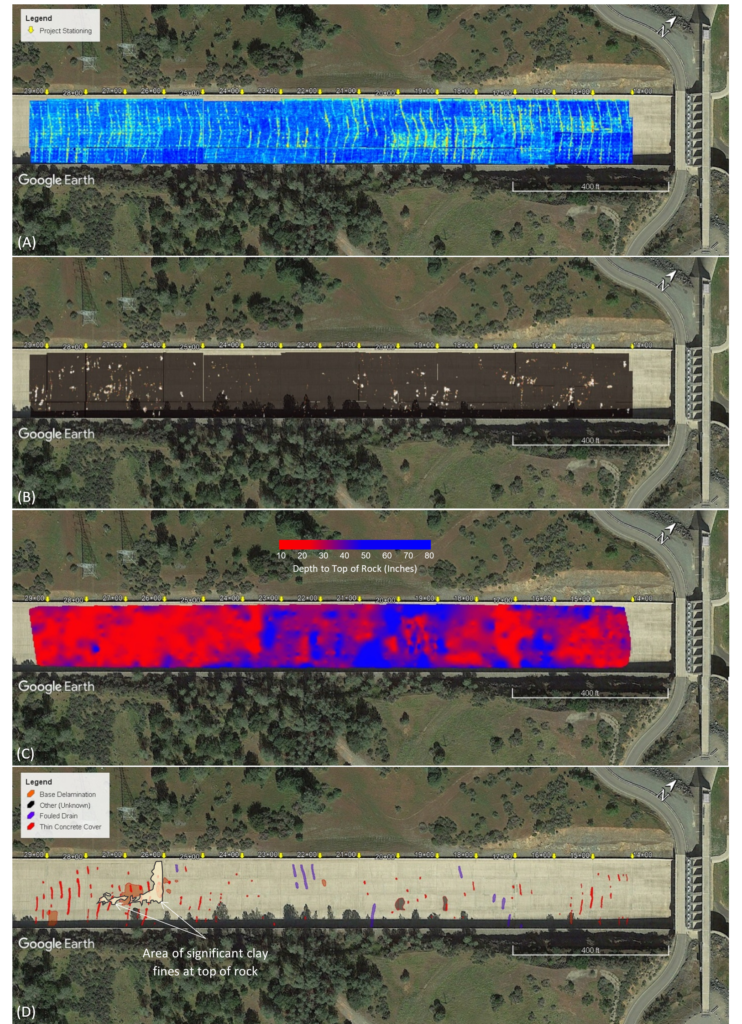
Figure 7. Plan view GPR maps produced for the Main Spillway. (A) Distribution of VCP drains and anchor mesh mats. (B) Discontinuities in bedrock below concrete. (C) Concrete thickness estimates. (D) Interpreted defects. Yellow arrows indicate project stationing. From Owen and Mallah (2017). See text for additional discussion.
the spillway slab (Figure 7B). Although it doesn’t provide orientation data for the discontinuities, we believed that the distribution of the observable features could assist with identification of probable shear zones or other regions of potential rock instability that might affect any preservation plans for the remaining spillway.
The lack of consistent reflection from the base of concrete complicated any effort to measure concrete thickness. However, with the information we had at hand regarding the placement of the base mesh at the rock anchors and the construction of the VCP drains, we could attempt to use those details as a proxy for the actual top of rock. Using depth estimates derived from the VCP drains and the anchor mesh, we produced a contour map of concrete thickness for the undamaged spillway (Figure 7C). Though we didn’t expect high accuracy with this approach, the GPR depth estimates nonetheless were useful in developing a general picture for concrete thickness distribution across the spillway, and yielded some interesting trends. We noted a zone of increased thickness approximately within the area between Station 19 and Station 23+50. That conclusion is supported by comparison of average concrete core thicknesses recovered during the emergency work (IFT, 2018, p. D-20). That information generally agrees with what we observe on the GPR data.
Caltrans’ final work product for this investigation was the summary interpretation of potentially significant anomalies (Figure 7D). Our interpretations centered on three general categories: regions of anomalously thin concrete, suspected fouled drains, and potential voids or delaminations between the base of the concrete and the top of rock.
Results indicated that most of the anomalously thin concrete cover is clustered below Station 24, where cores and GPR data indicate concrete is thinnest overall (Figure 8A). Corroboration of our most significant finding is that no voids were discovered beneath the spillway slab during destructive testing, consistent with what we saw on the GPR data. At Station 26, inspection of the rock surface where we identified a potential delaminated zone revealed a layer of fine-grained sand and silt between the slab and the top of rock (Figure 8B), confirming lack of bonding between concrete and bedrock at that location. Video inspection of the VCP drains at Station 22 revealed drain fouling at one location near the area where we interpreted potential fouling, but no significant sediment buildup in the drains was noted at the locations that we identified (Figure 8D).
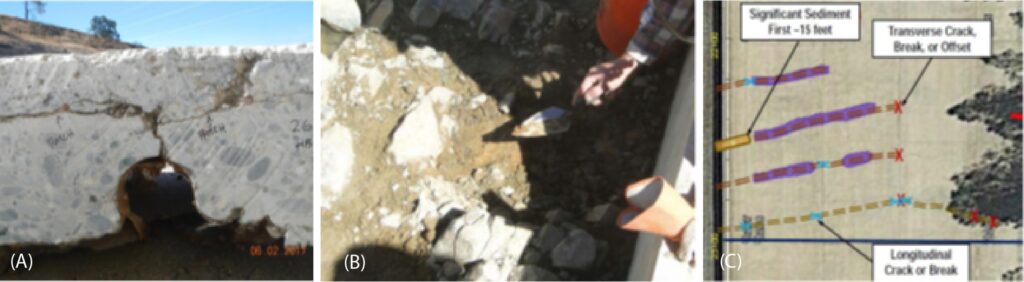
Figure 8. Results from destructive testing acquired during emergency response at the Main Spillway. (A) Thin concrete cover above a VCP drain in the vicinity of Station 26 (note lack of gravel filter blanket). (B) Sand and silt deposits above the bedrock surface in the vicinity of Station 26. (C) Results of video inspection of VCP drains at Station 22. From IFT (2018), Appendix D and Appendix I.
Emergency Spillway
At the Emergency Spillway, a secant (cutoff) wall and concrete splashpad were to be constructed at the spillway base to attenuate flow energy and mitigate future headcutting in the event of activation. GEOVision Geophysical Services was contracted to provide geophysical support for the design of that repair.
P-wave seismic refraction was chosen to delineate depth to competent bedrock and provide seismic velocities for qualitative evaluation of rock hardness. Line placement was completed “on-the-fly”, and final locations were dictated by on-site conditions at the time of data collection. P-wave seismic refraction was acquired along 20 profiles, each with 1 to 4 overlapping spreads of 48 geophones. Geophone spacing ranged from 4.5 to 10 ft and total line lengths were 211.5 to 1,125 ft. A 20-lb. sledgehammer or a truck-mounted 240-lb. accelerated weight drop (AWD) were used as the seismic sources. All lines were collected outside of the damaged spillway (Figure 9).
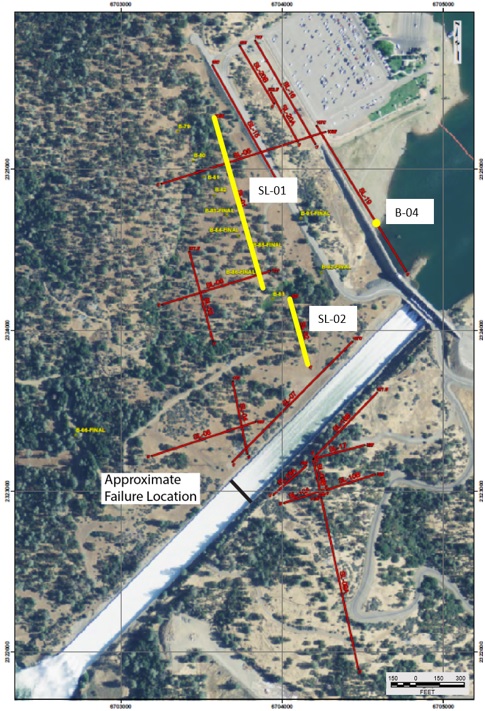
Figure 9. Locations of P-wave Seismic Refraction profiles and Borehole Geophysical logs. Highlighted locations (in yellow) shown in Figures 10-12. From Dalrymple (2017).
All profiles were processed using both unconstrained and constrained starting models using a local inversion tomography program. Unconstrained models were generated from 1D velocity profiles, and the constrained models were generated from time-term velocity sections. Both initial models were converted to 20-layer models and run for a minimum of 30 iterations. From the preliminary assessments of the subsurface conditions at the dam, it was expected that the geology consisted of variable weathered rock over competent rock. Tomography modeling was chosen for processing to allow for the variable weathering profiles to be more accurately rendered. Layer-based starting models were used to constrain initial conditions to preserve
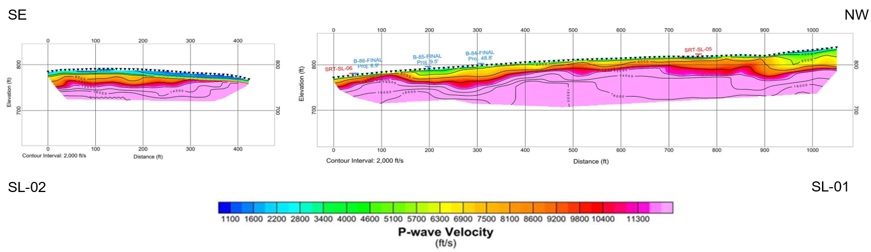
Figure 10. SRT-SL-01 and -02: Time-Term P-wave Seismic Tomography model, near the secant wall. Locations shown in Figure 9. View oriented looking downslope. Note that the weathered rock is thicker toward the northwest, with competent rock approaching the surface near the center and southeast of SL-01 and the northwest end of SL-02. From Dalrymple (2017).
subsurface layering, if present. In all cases, the unconstrained models and the constrained models were similar, confirming that velocity contrasts at the project site are derived from the observed deep and variable rock weathering, rather than well-defined bedding. The processed tomography models provided sections that showed the highly variable weathering profile as it transitions to more competent rock across the site. All P-wave velocity profiles showed similar results with variations in weathering thickness. An example time-term tomography section is shown as Figure 10.
Available geophysical and borehole logs from B-23, B-36, and B-81 were used to estimate the comparable velocity contours of the weathered rock and competent rock in the tomography models. Velocities of weathered rock were assumed to be in the range of about 5,000 ft/s to 7,000 ft/s and competent rock is assumed to be in the range of 9,000 to 11,000 ft/s. However, due to the gradational nature of the velocities of weathered and competent rock, alternate interpretations of the assumed velocity ranges are possible. Although no seismic lines were collected within the spillway, it was expected that the variable nature of the weathered section would have continued underneath the spillway, at least prior to construction.
Borehole geophysical logs were acquired at 95 locations across the project site. Boreholes were logged using a combination of Acoustic and Optical Televiewer and 3-Arm Mechanical Caliper logs. In situ velocity data were acquired at seven of the locations using a PS Suspension probe. Borehole logs were acquired as the drilling completed at each hole, and up to 10 drill rigs were simultaneously operated on site. That created a fast-paced, and dynamic geophysical logging schedule. To meet the demanding schedule, a logging crew remained on 24-hour standby at the site for approximately two months.
Interpretation of televiewer features was aided using core logs and other geophysical logs where available. Generally, televiewer feature interpretations aided by core data are more accurate than those derived from televiewer images alone. An example of an Acoustic Televiewer log with feature picks is provided as Figure 11.
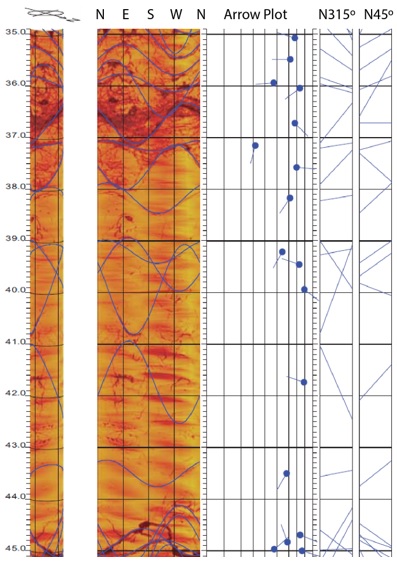
Figure 11. Borehole B-04, Acoustic Televiewer, 35 to 45 ft depth range. From Diehl (2018).
PS Suspension logging provided depth and velocity correlations to observed geologic conditions and other geophysical observations. An example PS Suspension log is provided as Figure 12. In the examples presented here, both the televiewer and PS log were from the same borehole located at the Emergency Spillway and coincident with a nearby seismic refraction line. Instances like this allowed for more detailed comparison and correlation between the geophysical observations and geologic logs.
The borehole geophysical logging, along with the seismic refraction surveys, provided rock depth, correlated rock types, and general conditions surrounding the spillway. Borehole logs that were in close proximity to the seismic refraction data showed good agreement, revealing a highly variable, weathered, and fractured rock profile throughout the site.
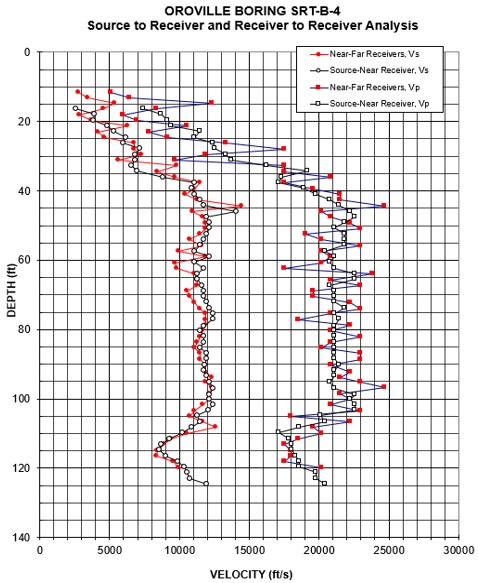
Figure 12. Borehole B-04, PS Suspension Log. Location shown in Figure 9. From Diehl (2018).
CONCLUSION
Safety is a long game, and no one can become complacent where it involves ensuring the integrity of the nation’s civil infrastructure.
So, how did geophysics contribute to understanding the spillway incident? The contributions can be summarized in four key points:
- It provided a better understanding of the as-built service condition of the spillway, including concrete thickness, drain placement, and anchor placement.
- It provided a better understanding of the variability of rock discontinuities at the Main Spillway and the Emergency Spillway.
- It provided data to assist with the design of both the Main Spillway and Emergency Spillway repair.
- It illustrated the important benefit of detailed, non-destructive testing for supplementing standard inspections and destructive testing for evaluating the characteristics and conditions of the spillways and their foundations.
The geophysical information provided additional confidence that allowed DWR engineers to determine that the remaining undamaged portion of the Main Spillway could be kept temporarily in service with high probability of success. The results also allowed DWR to proceed with immediate, initial construction and material removal in the Emergency Spillway area to prepare for final construction and repairs.
EPILOGUE
DWR contractors began rebuilding the Main and Emergency spillways in May 2017. Emergency repairs on the Main Spillway were completed in November 2017 to allow controlled discharges as needed to maintain safe reservoir levels. In 2018, the secant cutoff wall and splashpad were completed at the Emergency Spillway to mitigate future headcutting and erosion in the event of activation. By 2019, reconstruction of the Main Spillway to current design standards was completed (Figure 13). Grading of the eroded areas was completed in 2020. Revegetation of the damaged areas is ongoing.
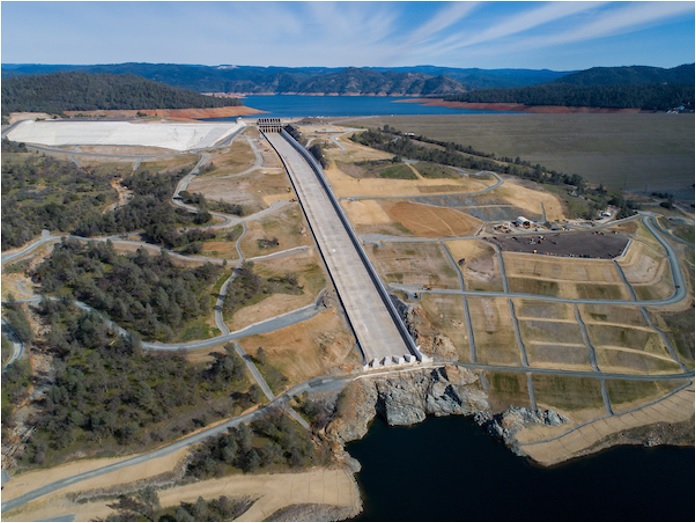
Figure 13. Oroville Dam Main Spillway and Emergency Spillway in 2020, after completion of reconstruction efforts.
DISCLAIMER
The California Department of Water Resources (DWR) did not participate in this article. All opinions presented herein are those of the authors. All supporting information presented herein is public-domain and available for independent review. Unless otherwise noted, all photos herein are reproduced courtesy of DWR and are available on their website at https://water.ca.gov/Programs/State-Water-Project/SWP-Facilities/Oroville/Oroville-Spillways
REFERENCES
Cole KA, McJunkin RD, 1978, Geology of the Lake Oroville area, Butte County, California: DWR Geological Maps, B 203-78.
Dalrymple WM, 2017, GEOVision report, Oroville Dam seismic refraction survey 17125-01: Report prepared for the California Department of Water Resources, DOE – Project Geology, Oroville Spillway Restoration Team, GEOVision Geophysical Services, Inc., 06/16/2017, 61 pages.
Diehl JG, 2018, GEOVision Report, Oroville Dam borehole geophysics 17101-01 rev 3: Report prepared for the California Department of Water Resources, DOE – Project Geology, Oroville Spillway Restoration Team, GEOVision Geophysical Services, Inc., 03/13/2018, 1568 pages.
DWR, 1965, Oroville dam spillway, chute drainage system: California Department Water Resources, State Water Facilities, Oroville Division, Spec. No. 65-09, Drawings A-3B9-1 through A3B9-4, Sheets 47-50.
IFT, 2018, Independent Forensic Team report, Oroville Dam spillway incident: Association of State Dam Safety Officials, Independent Forensic Team Report, January 5, 2018, https://damsafety.org/sites/default/files/files/Independent%20Forensic%20Team%20Report%20Final%2001-05-18.pdf
Owen W, and Mallah M, 2017, Mutual aid report, Oroville Dam spillway GPR investigation: Report prepared for the California Department of Water Resources Division of Operations and Maintenance, California Department of Transportation, Sacramento.
Saucedo GJ and Wagner DL (eds), 1992, Geologic map of the Chico quadrangle, California, 1:250,000: California Division Mines Geology Regional Map Series, Map No. 7A.
Author Bios
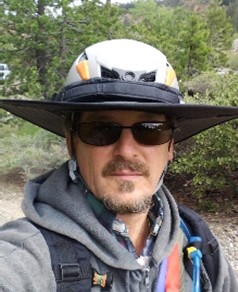
William Owen, PGP, CEG, PG
Bill Owen is a Professional Geophysicist and chief of the Geophysics and Geology Branch for the California Department of Transportation in Sacramento. He has over 30 years of experience managing and conducting engineering geophysical investigations for highway infrastructure throughout the state. He has also participated in numerous research projects and federal outreach efforts demonstrating the efficacy of geophysical methods for transportation and engineering problems.
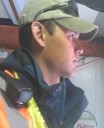
William Dalrymple, PGP
William Dalrymple is a Professional Geophysicist with the Geophysics and Geology Branch at the California Department of Transportation. He has 20 years experience, both private and public, conducting geophysical projects for highways, dams, mine sites, nuclear and chemical plants, and environmental sites worldwide. His current focus is on performing seismic, electrical, electromagnetic, and GPR investigations for transportation projects throughout California.
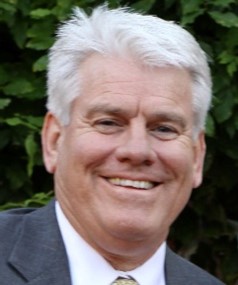
John Diehl, PE
John Diehl received his BSCE in 1976 from California State Polytechnic Institute of Pomona. His Masters in engineering was received in 1977 from UCLA, where he also attended to receive his MBA in 1988. With two partners, he founded GEOVision in 1995, which nearly 30 years later is one of the most respected geophysical consulting firms in the United States. In the course of his career, he has authored or co-authored over 30 publications, focusing primarily on geophysical instrumentation and geophysical testing. He is a lifetime member of ASCE and is a Professional Engineer in the state of California.